HACS
|文章标题:
Nutrients in the fate of pluripotent stem cells
【原文PDF文档领取方法】
复制英文标题,然后下拉到本文底部发送到后台|核心内容:
多能干细胞模拟了早期哺乳动物体外发育的某些特征。
培养基提供的营养物质可以影响多能干细胞的自我更新、谱系分化和最早的分化。
然而,哪些特定的营养物质支持这些不同的结果及其作用机制仍在积极研究中。
在这里,我们评估了关于影响多能干细胞命运的营养物质及其代谢转化的现有数据。
我们还讨论了在这个日益迅速扩大的基础和实际重要性的领域中有待研究的关键问题。 原文摘要:
Pluripotent stem cells model certain features of early mammalian development ex vivo.
Medium-supplied nutrients can influence self-renewal, lineage specification, and earliest differentiation of pluripotent stem cells.
However, which specific nutrients support these distinct outcomes, and their mechanisms of action, remain under active investigation.
Here, we evaluate the available data on nutrients and their metabolic conversion that influence pluripotent stem cell fates.
We also discuss key questions open for investigation in this rapidly expanding area of increasing fundamental and practical importance.
INTRODUCTION
Pluripotent stem cells (PSCs) self-renew or, with specific cues, can differentiate into the three primary germ lineages in vitro. A defining feature of pluripotency is remarkable plasticity in cell identity, leading to potential applications in tissue engineering, regenerative medicine, and studies of early embryonic development (Smith, 2017; Tsogtbaatar et al., 2020).
In considering the fate of PSCs, we define pluripotent/self-renewing stem cells as cells that express core pluripotency transcription factors including OCT4, NANOG, SOX2, and others, and differentiation as the silencing of core pluripotency transcription factors coupled with the gain of lineage-specifying biomarkers (Yeo and Ng, 2013).
Functionally, two principal hallmarks that define PSCs are blastocyst chimerism and teratoma formation, which test in vivo ability to re-enter development in a host embryo and spontaneous generation of the three germ layers, respectively (De Los Angeles et al., 2015).
Of particular interest here are studies suggesting that supplied nutrients, with or without intracellular processing, have active, guiding roles in PSC identity and cell fate transitions (Intlekofer and Finley, 2019; ShyhChang and Ng, 2017; Tsogtbaatar et al., 2020).
For example, the metabolic flux of nutrients through glycolysis, the tricarboxylic acid (TCA) cycle, and one-carbon metabolism generates intermediate metabolites used in reactions to modify the PSC epigenome, chromatin structure, and gene expression (Carey et al., 2015; Moussaieff et al., 2015; Shiraki et al., 2014; Shyh-Chang et al., 2013b; TeSlaa et al., 2016).
In addition to studies linking PSC-produced intermediate metabolites with epigenome modifications, many interesting questions remain regarding the role of nutrients, including sugars, amino acids, lipids, and others, in the control of PSC fate.
For example, recent results suggest that nutrients can also influence PSC fate through mechanisms that do not include metabolic flux through anabolic or catabolic pathways (Chi et al., 2020; Cornacchia et al., 2019; Na et al., 2020; Song et al., 2019; Vardhana et al., 2019; Zhu et al., 2020).
One potential interpretation of these studies is that specific nutrients signal conditions in the microenvironment prior to, or concomitant with, triggers that control PSC fate.
In this perspective, we examine the contributions of nutrients in PSC fate through both metabolic conversion and nutrient-triggered signaling.
Figure 1. Nutrient sensing pathways impact pluripotent stem cell fate
Nutrient-specific sensing occurs via the hexosamine biosynthesis pathway (HBP, gray), mTORC1 (blue), and AMPK (yellow).
HBP integrates carbohydrates (glucose), amino acids (glutamine), lipids (acetyl-CoA), and nucleotides (UTP), culminating in the generation of UDP-GlcNAC, a substrate for O-GlcNAcylation reactions by OGA/OGT.
Signaling by mTORC1 and AMPK is regulated by concentrations of glucose and amino acids at multiple sensors, with activities localized to the lysosomal membrane.
These specific nutrients fuel enzyme-based alterations in the epigenome, gene transcription, translation, and PTMs to shape PSC fate (Table 2).
Glucose-6-phosphate, G6P; fructose-6-phosphate, F6P; glutamine-fructose-6-phosphate transaminase, GFAT; fructose 1,6-bisphosphate, FBP; endoplasmic reticulum, ER; O-GlcNAc transferase, OGT; O-GlcNAcase, OGA; dihydroxyacetone phosphate, DHAP.
Intracellular(situated or occurring inside a cell or cells) pyruvate routing can occur to
(1) produce lactate via lactate dehydrogenase (LDH) with NAD+ generated to sustain glycolytic flux,
(2) produce acetyl-CoA via pyruvate dehydrogenase (PDH) for TCA cycle oxidation,
(3) produce oxaloacetate via pyruvate carboxylase (PCB) to replenish TCA cycle intermediates, and
(4) localize to the nucleus to regulate the epigenome (Figure 1) (Sutendra et al., 2014).
PDH inhibition, which blocks pyruvate entrance into the TCA cycle, supports mouse iPSC reprogramming and viability, whereas increased pyruvate flux into the mitochondria decreases pluripotency biomarker expression, reduces PSC self-renewal, and lowers PSC viability (Rodrigues et al., 2015; Zhang et al., 2017a).
Upon differentiation, PDH gene expression rapidly decreases, but PDH protein levels stay the same.
By contrast, ATP-citrate lyase (ACYL) and acetyl-CoA synthetase (ACCS2), which provide pyruvatederived acetyl-CoA for histone acetylation reactions that support pluripotency, rapidly decrease upon differentiation (Moussaieff et al., 2015).
This fate-dependent pattern of metabolism enzyme expression suggests that, upon pluripotency exit, the hierarchy of pyruvate routing shifts toward mitochondrial TCA cycle oxidation to support PSC differentiation.
mTOR in pluripotency
Mammalian target of rapamycin (mTOR) complex 1 (mTORC1) is a serine/threonine protein kinase whose activation in response to specific nutrient levels phosphorylates downstream substrates that regulate cell growth, proliferation, and survival (Saxton and Sabatini, 2017).
An amino acid-mediated interaction between the Rag family of guanosine triphosphatases (GTPases) and mTORC1 causes mTORC1 re-localization from the cytosol to the lysosomal membrane (Kim et al., 2008; Sancak et al., 2008).
Specific amino acid-responsive upstream regulators of mTORC1, including lysosomal vacuolar H(+)-adenosine triphosphatase (v-ATPase), SAM sensors, leucine sensor Sestrin2, arginine sensor CASTOR1, SLC38A9, and SAMTOR (Chantranupong et al., 2016; Gu et al., 2017; Wang et al., 2015; Wolfson et al., 2016; Ye et al., 2015), signal to the Rag GTPases to activate or inactivate mTORC1 (Figure 1; Table 2).
Similar to amino acids arginine, leucine, and glutamine, glucose can also directly activate mTORC1.
In glucose deprivation studies, mouse embryonic fibroblasts (MEFs) showed a decrease in mTORC1 kinase activation (Kalender et al., 2010). Mechanistically, glucose controls mTORC1 activity by influencing the binding of the v-ATPase to the Ragulator, a complex of five LAMTOR proteins that associates with the Rag GTPases (Figure 1) in order to activate mTORC1 (Efeyan et al., 2013).
Additional recent studies showed intracellular glucose detection by mTORC1 occurs through a glucose-derived metabolite, dihydroxyacetone (DHAP). In glucose-starved conditions with decreased mTOR activity, the synthesis of DHAP restored mTOR activity (Orozco et al., 2020).
In sum, mTORC1 activation occurs by several distinct amino acid and glucose signaling mechanisms, which aligns with its central role as a nutrient sensor and actuator of cell growth, proliferation, and survival (Figure 1; Table 2).
In conditions of nutrient abundance, mTORC1 binds ULK1 to inhibit autophagy, and conversely, nutrient starvation dissociates mTORC1 from ULK1, triggering autophagosomes to form (Rabinowitz and White, 2010).
In hPSCs, nutrient starvation inhibited mTORC1 activation, increased autophagosome formation, and decreased levels of OCT4, SOX2, and NANOG transcription factors (Cho et al., 2014; Zhou et al., 2009).
Autophagy inhibition in starvation conditions caused pluripotency transcription factors to accumulate, suggesting a role for nutrients in controlling pluripotency by regulating autophagy (Cho et al., 2014). Immunofluorescence microscopy and immunogold-stained electron microscopy during nutrient starvation confirmed pluripotency transcription factor interactions with autophagosomes (Cho et al., 2014), suggesting altogether that loss of pluripotency from nutrient starvation occurs through an mTORC1-autophagy response.
Amino acid deprivation is the most studied trigger for mTORC1 inhibition and subsequent induction of autophagy (Saxton and Sabatini, 2017).
Amino acids such as glutamine, methionine, and threonine have demonstrated roles in pluripotency maintenance (Carey et al., 2015; Shiraki et al., 2014; Shyh-Chang et al., 2013b; Vardhana et al., 2019) and may promote pluripotency by signaling nutrient abundance to activate mTORC1.
For example, threonine is a positive regulator of mESC self-renewal (Han et al., 2013; Shyh-Chang et al., 2013b; Wang et al., 2009). Culture medium deficient in threonine, but not in any other amino acid, caused a decrease in alkalinephosphatase-positive mESC colonies (Wang et al., 2009).
Threonine dehydrogenase (TDH), the rate-limiting enzyme in threonine catabolism, supports somatic cell reprogramming into mouse iPSCs because TDH knockdown decreased reprogramming efficiency (Han et al., 2013).
Threonine supports pluripotency by maintaining histone methylation levels (Shyh-Chang et al., 2013b; Wang et al., 2009) and by regulating mTORC1 activity (Ryu and Han, 2011).
mESCs incubated with threonine increased expression of pluripotency proteins OCT4, NANOG, and SOX2 (Ryu and Han, 2011).
Inhibition of mTORC1 signaling activity with rapamycin abolished this effect, further suggesting a role for nutrient-activated mTORC1 in regulating pluripotency (Ryu and Han, 2011).
It is possible that threonine promotes mESC self-renewal by keeping mTORC1 activity below the level that triggers autophagy and degradation of pluripotency transcription factors.
Future studies could identify whether other nutrients, such as glucose, or specific amino acids, such as proline, also sustain pluripotency through mTORC1 inhibition of autophagy.
Of course, mTOR effects on transcription, translation, and metabolism could also influence pluripotency.
For example, nutrient starvation could inhibit mTORC1 activity and reduce translation of pluripotency-related transcripts.
It is important to note that contradicting studies indicate that an autophagy response can promote instead of inhibit pluripotency. For example, FOXO1, a master regulator of core autophagy genes, maintains high autophagic flux to maintain mESC pluripotency, likely by generating substrates that support rapid cell proliferation (Liu et al., 2017).
Similarly, PINK1/OPTNmediated mitophagy is important for clearing depolarized mitochondria in order to maintain mitochondrial homeostasis that appears critical for pluripotency (Wang et al., 2021a).
A possible explanation for these contradictory results is that a precise balance of autophagic activities—neither complete activation nor complete inhibition—is required to maintain pluripotency.
mTOR complex activity is also implicated in a process that suspends, or reversibly halts, development, called diapause (Bulut-Karslioglu et al., 2016; Hussein et al., 2020).
Preimplantation mammalian embryos can survive ex vivo for up to 2 days, but deprivation of glucose, arginine, and leucine can induce diapause (Gwatkin, 1966; Naeslund, 1979).
The Bulut-Karslioglu group hypothesized that inhibiting growth pathways could replicate this nutrient-starved diapause state. Their work identified mTOR as a regulator of developmental timing and pluripotency, determining that mTOR inhibition could induce a diapause state that significantly prolonged pluripotency.
Specifically, nutrient starvation activates the serine-threonine liver kinase B1 (LKB1)-AMPK signal transduction pathway, which inhibits mTORC2 to cause diapause (Hussein et al., 2020). While these studies suggest that nutrient-regulated mTORC2 is a primary player in controlling diapause and prolonging pluripotency, mTORC2 has been documented to additionally regulate mTORC1 activity (Szwed et al., 2021), making it possible that mTORC1 may also play a role in this phenomenon.
A role for nutrients in mTORC1 regulation of pluripotency exit and subsequent differentiation is less clear. Inhibition of mTORC1 during germ lineage non-directed embryoid body formation increased mesoderm and endoderm differentiation and decreased ectoderm differentiation (Jung et al., 2016; Zhou et al., 2009).
Chemical screens with additional mTORC1 inhibitors confirmed this result (Nazareth et al., 2016).
Furthermore, mouse embryos containing a loss-of-function mutation in mTOR showed malformations in the ectoderm-derived telencephalon with subsequent loss of viability (Hentges et al., 2001).
Similarly, a loss-of-function mutation in TSC2, an inhibitor of mTOR, also caused embryonic lethality due to overgrowth of ectoderm-derived neuroepithelium (Rennebeck et al., 1998).
These studies combined highlight a role for mTORC1 activity in promoting ectoderm differentiation.
Because mTORC1 responds to many different nutrients, it will be interesting to determine whether a particular nutrient can promote lineage differentiation through mTORC1 activated signaling, or whether a combination of nutrients will be necessary for this effect.
参考文献:Nutrients in the fate of pluripotent stem cells;
Vivian Lu,1,8 Irena J. Roy,2,8 and Michael A. Teitell3,4,5,6,7,* 1Department of Molecular and Medical Pharmacology, David Geffen School of Medicine, University of California, Los Angeles, Los Angeles, CA 90095, USA 2Developmental and Stem Cell Biology, School of Medicine, University of California, San Francisco, San Francisco, CA 94143, USA 3Department of Pathology and Laboratory Medicine, David Geffen School of Medicine, University of California, Los Angeles, Los Angeles, CA 90095, USA 4Department of Pediatrics, David Geffen School of Medicine, University of California, Los Angeles, Los Angeles, CA 90095, USA 5Molecular Biology Institute, University of California, Los Angeles, Los Angeles, CA 90095, USA 6Department of Bioengineering, University of California, Los Angeles, Los Angeles, CA 90095, USA 7Jonsson Comprehensive Cancer Center, California NanoSystems Institute, and Broad Center for Regenerative Medicine and Stem Cell Research, University of California, Los Angeles, Los Angeles, CA 90095, USA 8These authors contributed equally *Correspondence: mteitell@mednet.ucla.edu https://doi.org/10.1016/j.cmet.2021.09.013
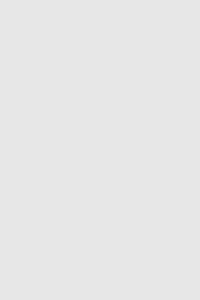
你知道吗?点赞后系统自动给你推荐类似优质原创文章哦!
底部是系统推送的广告,与本文内容无关